Our Blog
Geo-Electrical Resistivity meter
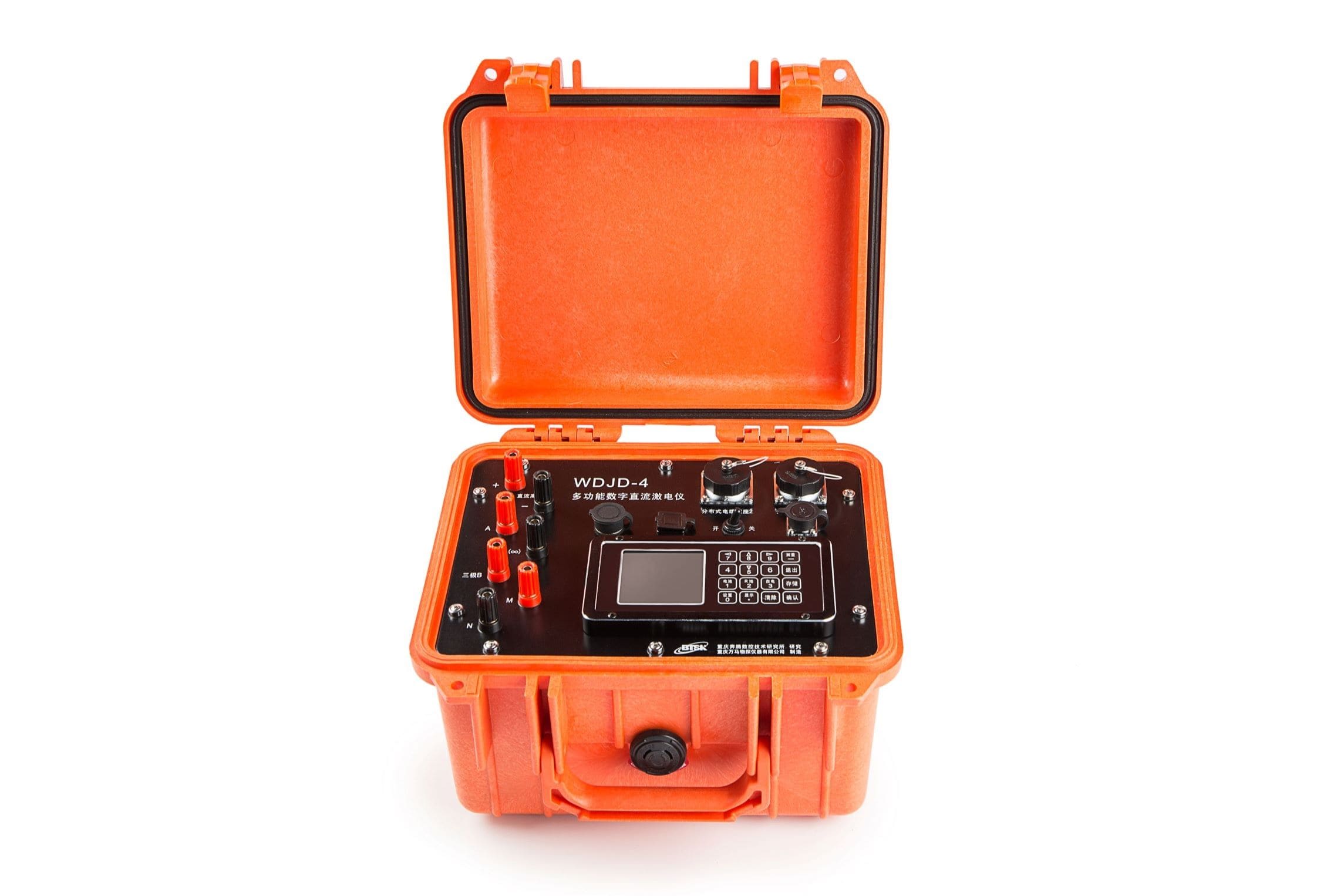
In simple geoelectrical resistivity meter is nothing but surveying equipment that helps determine the subsurface resistivity by measuring the earth's surface. It is also helpful for examining the groundwater, mineral prospects, and other civil engineering applications. To know this in detail, let us get to know what geoelectric resistivity is and how it works.
Explore MoreEarth Resistivity Tomography
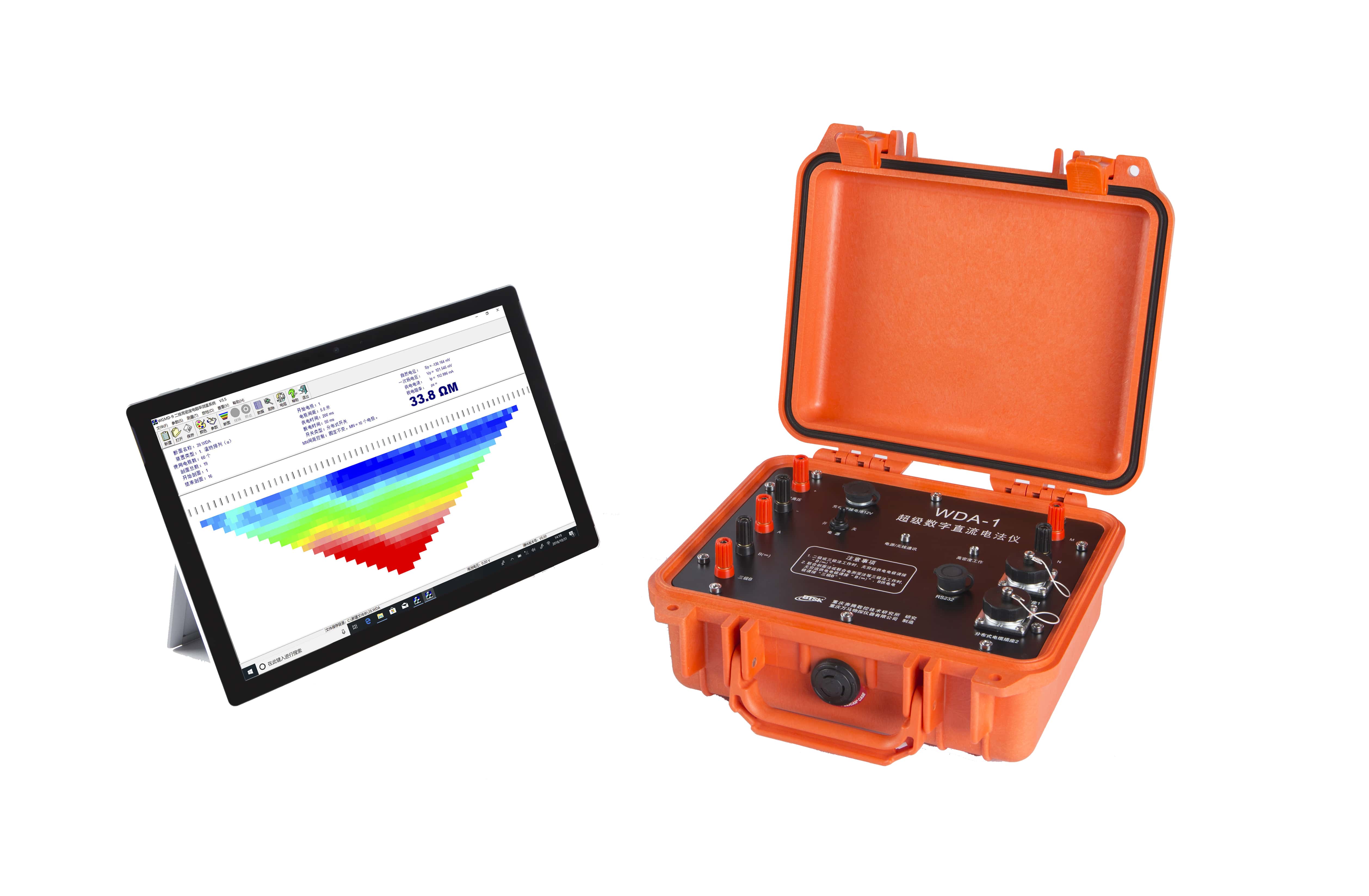
Electrical resistivity tomography (ERT) is a well-known subsurface-imaging geophysical technique and is widely applied to mineral prospecting, hydrological exploration, environmental investigation, and civil engineering, as well as archaeological mapping.
Explore MoreGround Water Detector
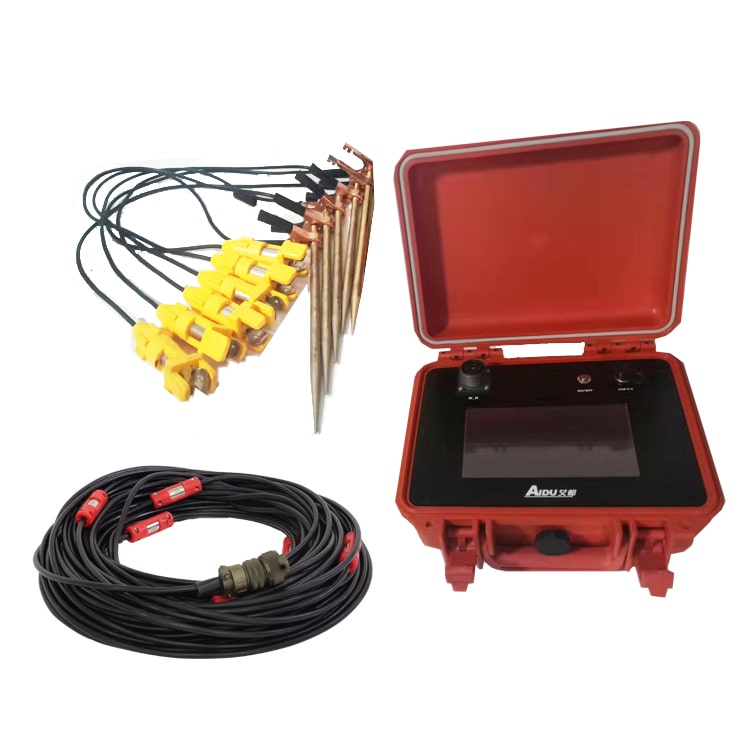
As we all know, groundwater is an invisible and inevitable resource that is indeed for every human in the globe around. However, it has been really tough these days for groundwater exploration as groundwater depletion is alarming everywhere. Let's get in detail about the following in this blog that gives you the knowledge of groundwater.
Explore MoreHow do seismographs record earthquakes?
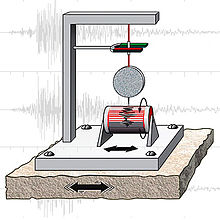
Whenever we hear news about an earthquake in any part of the world, the following phrase or word will be about the earthquake's intensity to know what damage it caused. Seismographs are the indicator that helps you know
Explore MoreGeophone Seismic Survey
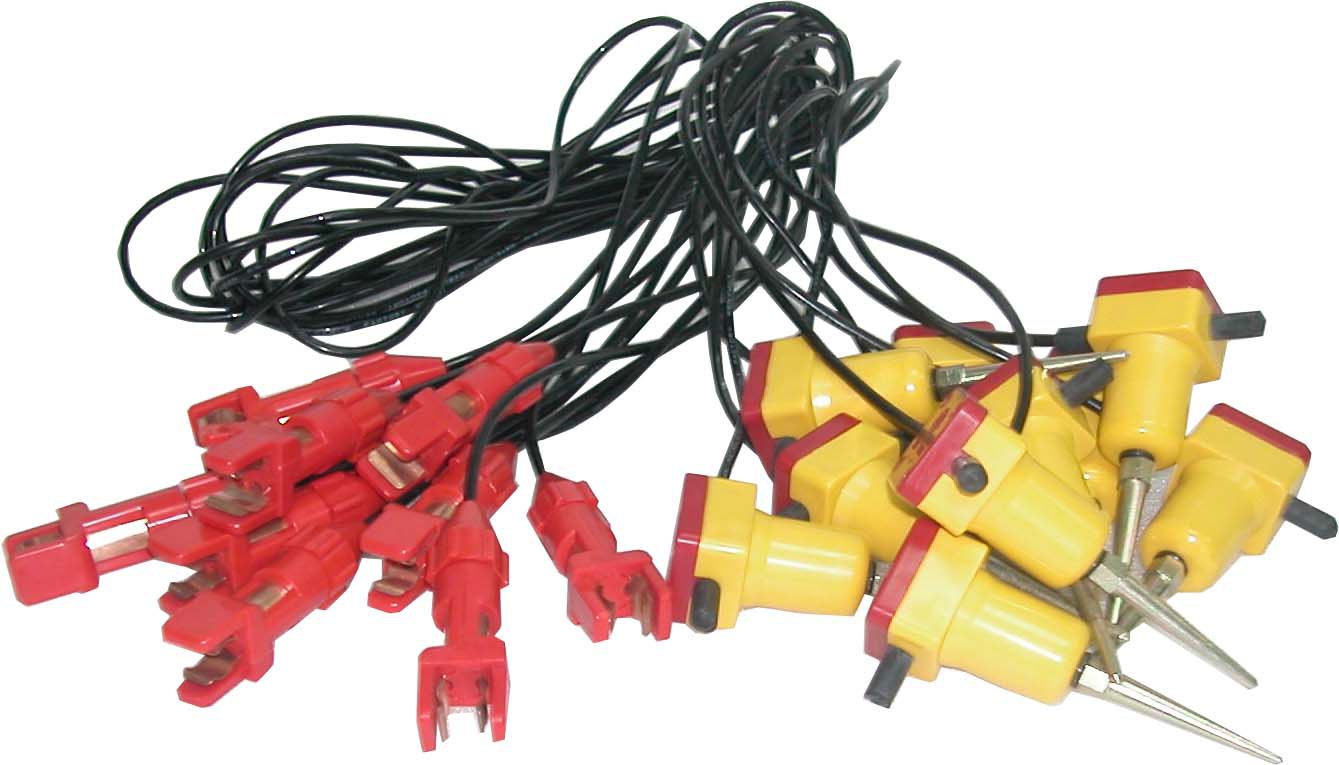
A geophone is a transducer that converts ground movement (velocity) into voltage, which may be recorded at a recording station. The deviation of this measured voltage from the baseline is called the seismic response and is analyzed for the earth's structure.
Explore MoreGeophysical Borehole Logging Equipment
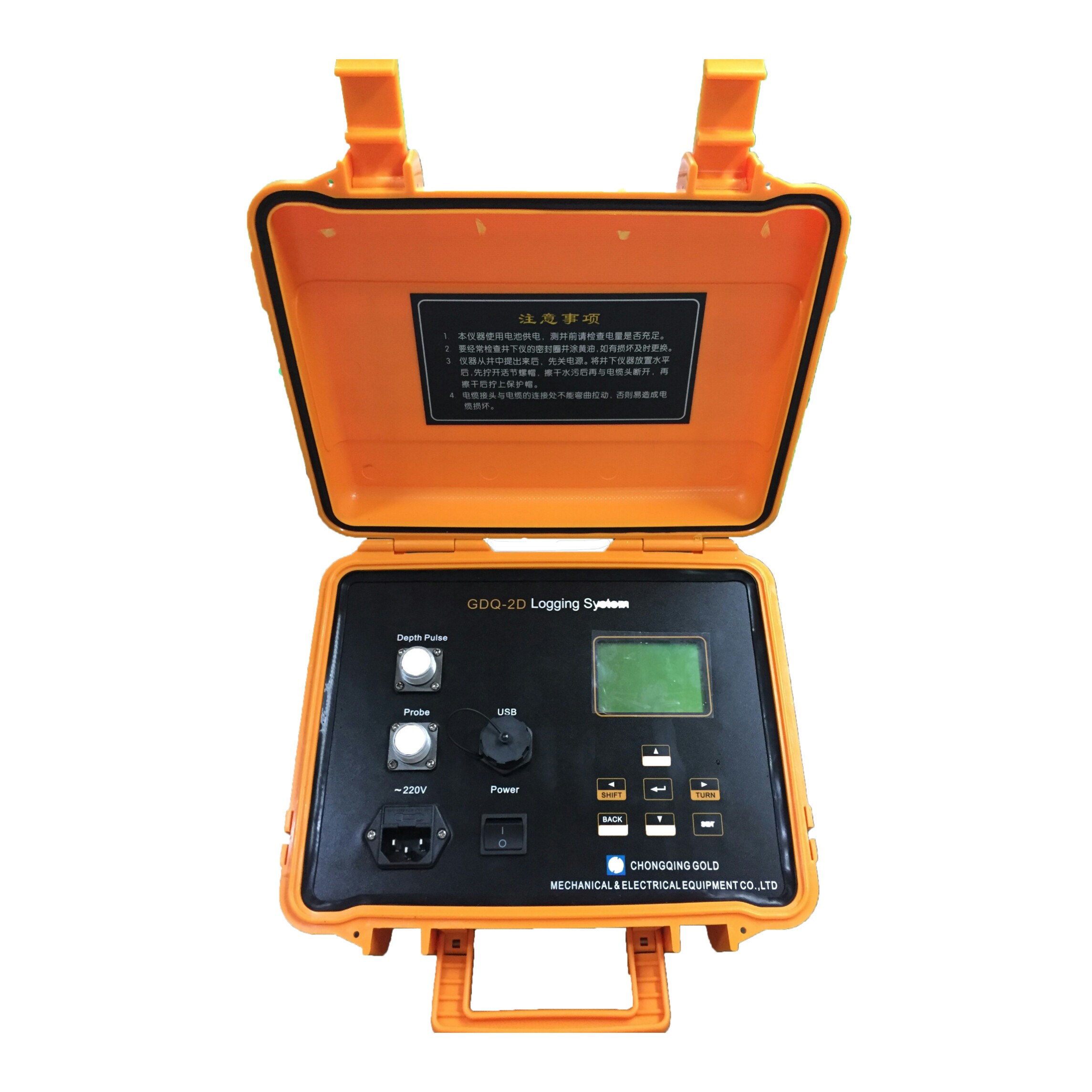
Geo-science industries require reliable pieces of equipment to measure accurate and objective results in even the most demanding conditions. When it comes to your own work or project, you must invest in the most effective systems and machines to minimize costs and maximize the benefits. That’s where WTS geophysical borehole logging Equipment comes in.
Explore MoreTransmission Electron Microscopes
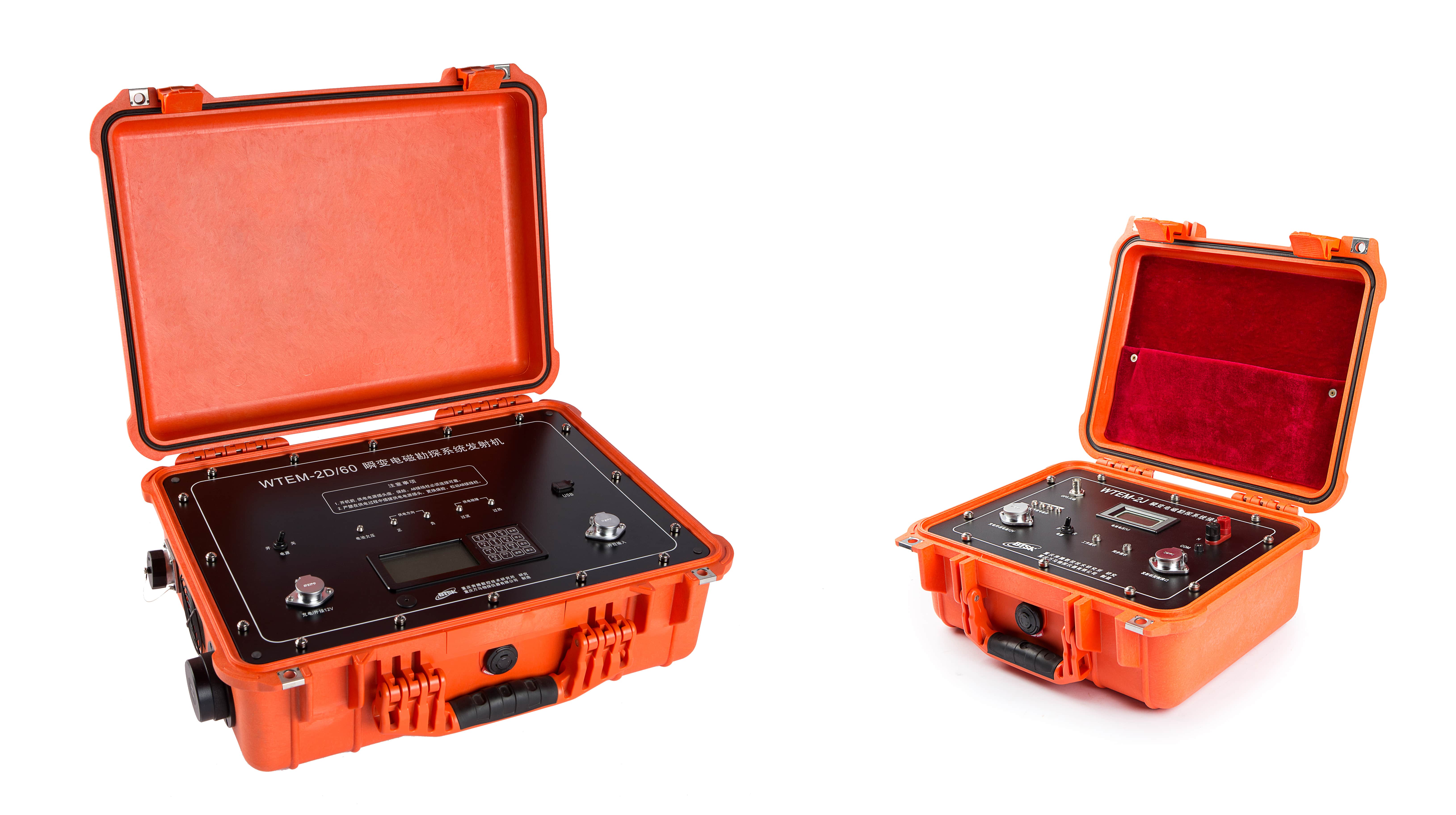
Transmission Electron Microscope was the initial type of Electron Microscope to be developed and is patterned precisely on the light transmission except that a focused beam of electrons is used instead of light to “see-through” the specimen.
Explore More